The Role of Noradrenaline in Energy Homeostasis
Volltext
Abbildung
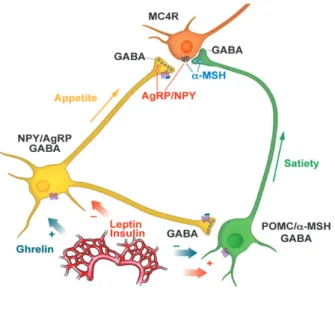
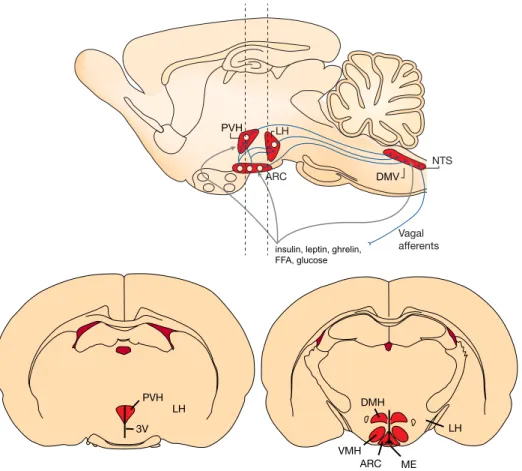
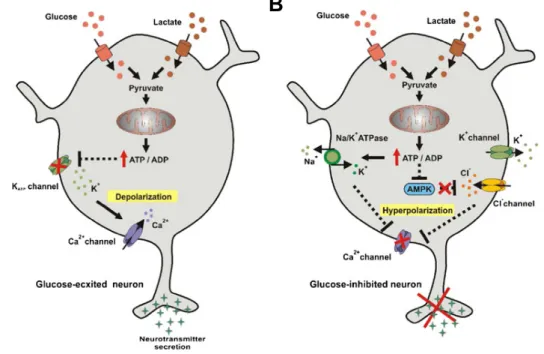
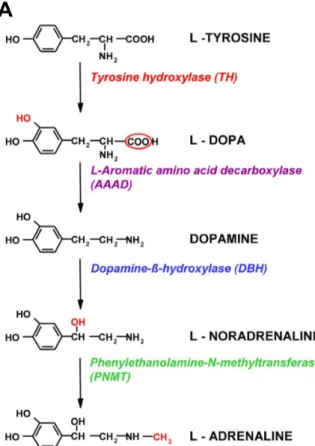
ÄHNLICHE DOKUMENTE
We thus aimed to study a potentialfunctional interaction between Rhcg andthe multi-unit protein vacuolar H + -ATPase (H + -ATPase), which constitutes the main H +
In the setting of congestive heart failure, mRNA expression levels of ANP and BNP in cardiomyocytes are markedly increased and plasma ANP concentrations
Consistent with this result, we observed a rise of ATP levels produced by complex V, the final OXPHOS enzyme, in GBE-treated control and APP cells (Fig. Taken together,
Analysis using tetraploid aggregation indicates that a defect in S6K1 -/- placental trophoblast cells leads to growth retardation and developmental delay phenotypes of S6K1 -/-
While behavioral rhythms are regulated by a central circadian pacemaker, accumulating evidence suggests that peripheral clocks strongly contribute to the regulation
Kryazhimskii, A., Watanabe, Ch., and Tou, Y., 2002, The reachability of techno- labor homeostasis via regulation of investments in labor and R&D: a model-based analysis,
To address the mechanism involved in signaling of Gpr111 as well as effects of Gpr111 depletion on in vitro differentiation, brown and white adipocytes were isolated from
Figure 35 Histological analysis of adipose tissue of Ednra AT-KO animals and their corresponding Ednra floxed littermates after sustained cold exposure for one week Panel of