Measurement of charm fragmentation ratios and fractions in photoproduction at HERA
Volltext
Abbildung

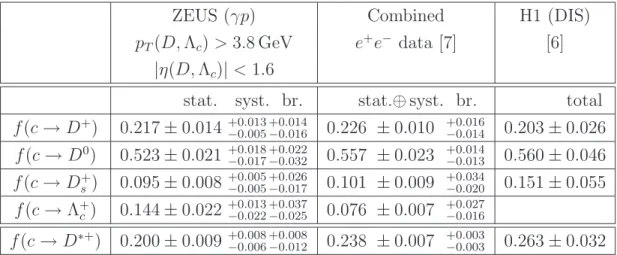
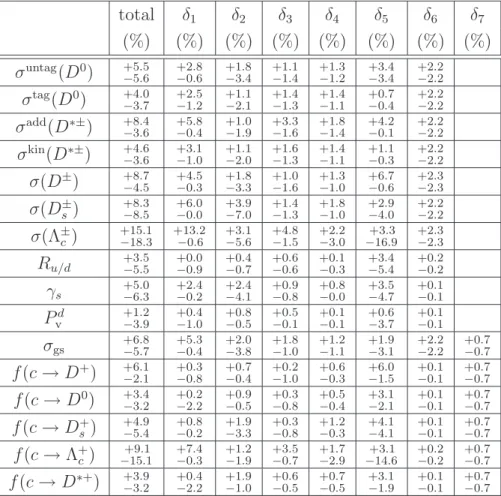
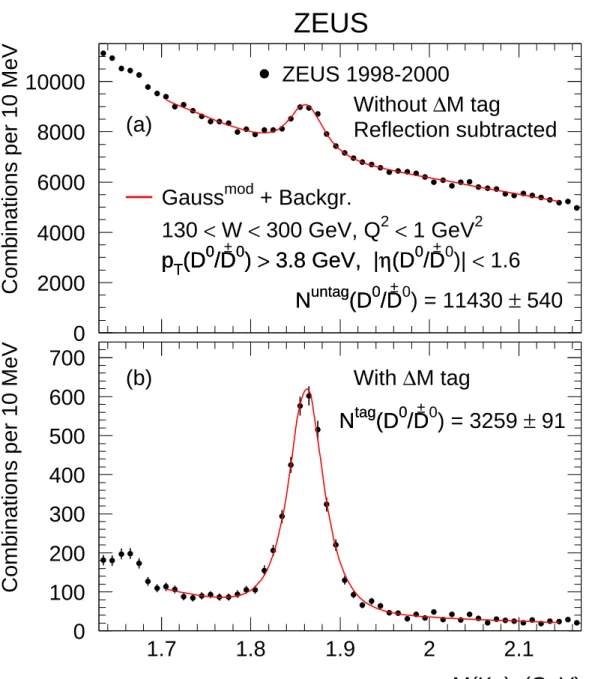
ÄHNLICHE DOKUMENTE
2 This, as Keynes has noted, was clearly perceived 300 years ago by Bernard Mandeville in his 1714 Fable of the Bees.
In order to determine the validity range of an uncorrelated hadron resonance gas model description of the open charm sector of QCD, without using details of the open charm
Although the quark masses are not tuned to the physical values matching K mesons, and the results correspond to decays of D s to pseudoscalar strange mesons, we attempt to compare
A 2011 Chinese white paper on foreign aid – groundbreaking in its own way for publicly discussing Chinese ODA policies and data – states that the purpose of China’s foreign aid is
We can use the molar volume to calculate substance amounts and volumes of gaseous reactants and products3. Calculate the volume of chlorine gas that reacts with 15 g
Floor / Newdecò Palladian Light 9090 Wall / Newdecò
A covariant generalization of the one- dimensional cascade model for quark fragmentation functions is presented, so as to include the transverse momentum behaviour
The results presented provide a more stringent test of QCD predictions compared to [ 1 ] because the transverse momentum distribution is sampled in finer bins - with smaller