liver injury and cancer
Volltext
Abbildung
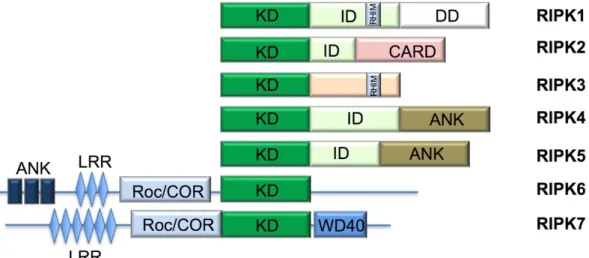
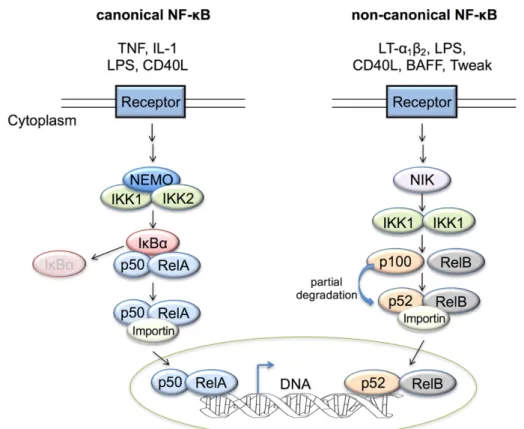
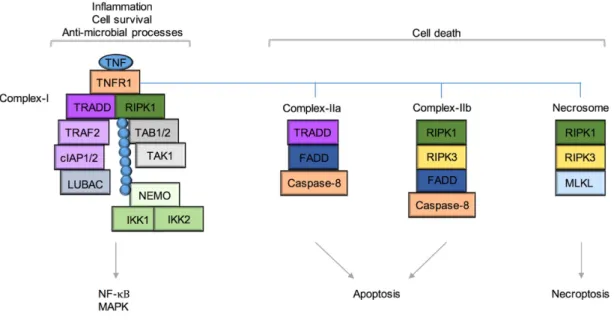
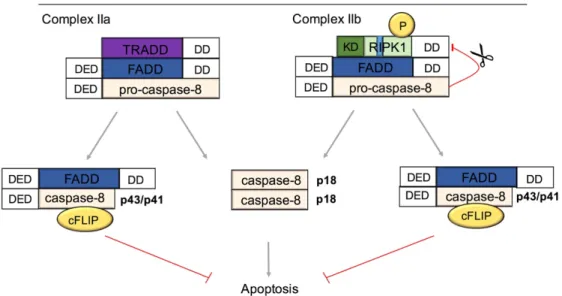
ÄHNLICHE DOKUMENTE
Improved understanding of the immune cell-mediated mechanisms involved in hepatocyte cell death could be beneficial for the development of common therapeutic strategies
In conclusion, from this current study, by using PrPC knockout mice, we showed a critical role of PrPC in the liver of aging mice by regulating the glucose/lipid metabolism, which
In a sensitivity analysis including only patients with liver imag- ing > 30 days before onset of COVID-19, imaging evidence of hepatic steatosis remained associated with
A New Flavonoid C-Glycoside from Solanum elaeagnifolium with Hepatoprotective and Curative Activities against Paracetamol- Induced Liver Injury in Mice..
There was a signifi cant (P < 0.05) decrease in the protein content in the livers of the paracetamol-treated group compared to the control group, while the groups treated
Taken together this study shows that the liver endothelial layer, mainly LSE Cs, represent a direct target of the cytotoxic effect of paracetamol and that activation of
Synergistic induction of cell death by TRAIL and chemotherapeutics has been described in different tumors cell Iines.6-8,14 Similarly, we have previously reported
Consequently, there is an urgent need for liver models that allow to screen drug candidates for their potential to induce idiosyncratic DILI in the early non- clinical