Molecular characterization of CNS interneurons:
Volltext
Abbildung
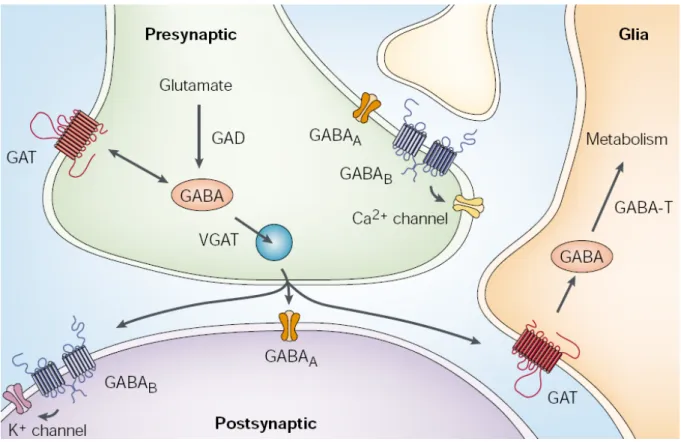
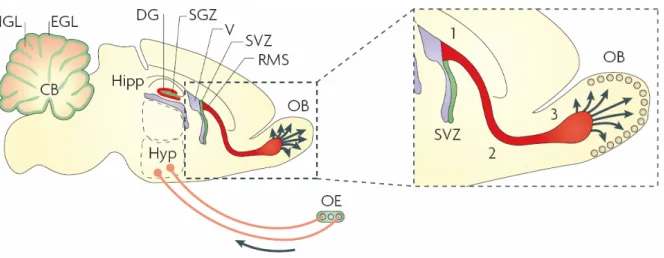
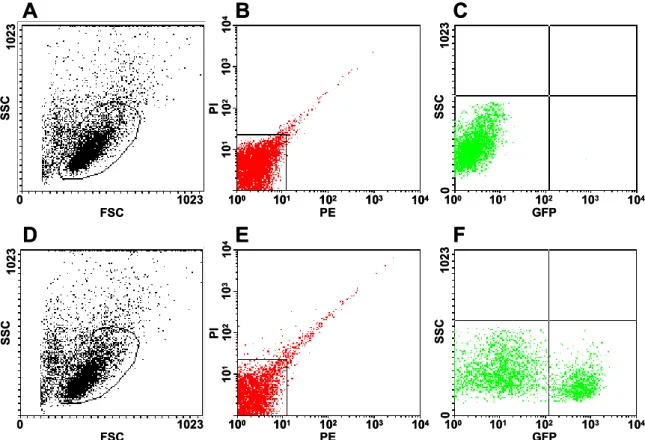
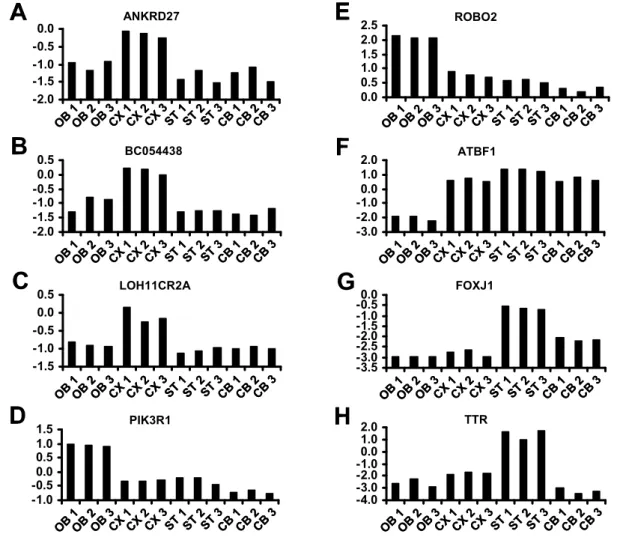
ÄHNLICHE DOKUMENTE
Electrical stimulation in the granule cell layer or outer molecular layer (ML) were used to target perisomatic or dendritic inputs respectively in the presence of NBQX and APV. This
After conditional depletion, CNS-resident microglia, but not peripheral monocytes, repopulated the brain within 5 days by extensive proliferation involving
developments have been used successfully to combine in vivo two-photon calcium imaging with subsequent dense reconstruction of neuronal circuits from complete 3D electron
On the basis of our findings that VM neuronal activity encodes sweet taste information and that the presynaptic terminals of VM neurons are in close proximity to cell bodies of
A more recent study in rats has confirmed the existence of D2 receptors on the afferent terminals by electron- microscopy using immunogold labeling (Gutièrrez-Mecinas et al,
Considering the prominent role of polySia for the migration of postnatally generated olfactory interneurons from the SVZ toward the olfactory bulb (Rutishauser, 2008),
Motion in the local antipreferred direction led to strong decreases in the local calcium signals, but the relation of the amplitude of increases and decreases was inhomoge- neous
These compu- tations comprise temporal frequency filtering by dendritic spines, improvement of the signal-to-noise ratio by adjusting the spatial sensitivity