Electric Power Generation, Specific Capital Cost, and Specific Power for Advanced Geothermal Systems (AGS)
Volltext
Abbildung
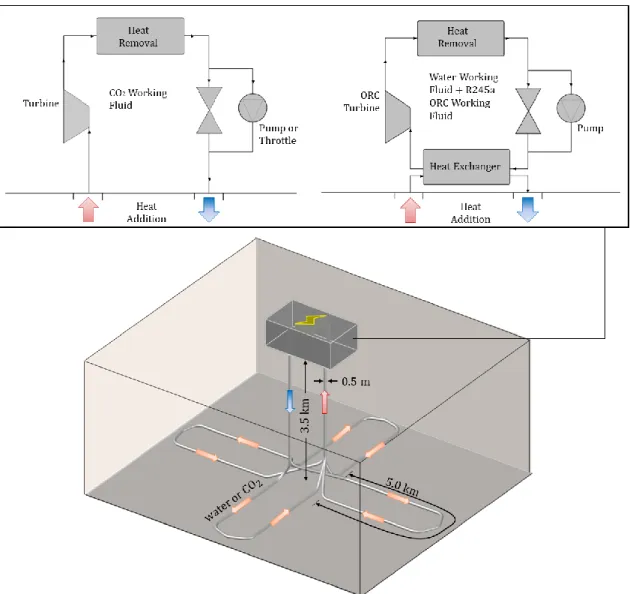
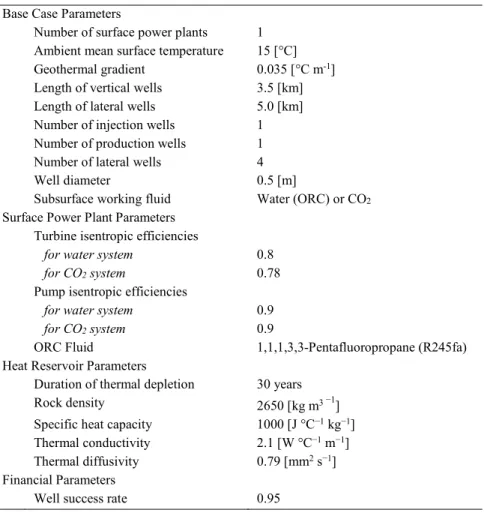

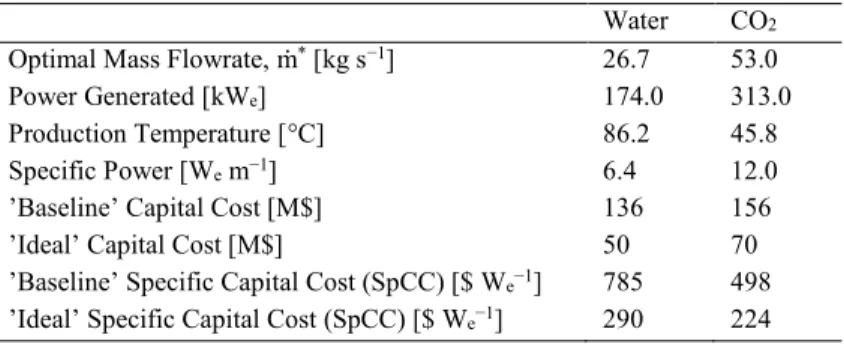
ÄHNLICHE DOKUMENTE
In case of typical geothermal conditions of the Upper Rhine Rift Valley with a geothermal water temperature of 160 °C and a mass flow rate of 65 kg/s, second law efficiency for
Proposition 1 If training contains only general human capital, then the mobility de- cision of workers is unaected if workers are paid their outside option (the competitive case) or
Based on the performed analysis of mathematical models for minimizing the power shortage, as used in domestic and foreign software packages to assess the adequacy of the EPS, we
Selection of the optimal portfolios of security measures that reduce the susceptibility of power grids to cyber
The expected energy exchange between cooperating systems is an important infor- mation supporting capacity expansion planning for electric power systems.. A model based
The development of a power infrastructure requires moving from an isolated national power supply system to the creation of trans-border power communications by means of coordinated
To quantify the potential benefits of real-time pricing in integrating plug-in hybrid electric vehicles into the electric grid, we will compare various PHEV charging schemes
Investigating the impact of plug-in electric vehicle charging on power distribution systems with the integrated modeling and simulation of transportation network. In Conference and