Microglia as therapeutic targets in retinal degeneration: role of translocator protein (18 κDa) (TSPO) and minocycline
Volltext
Abbildung
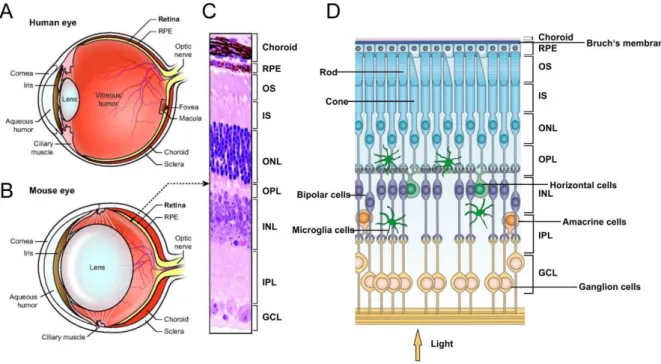
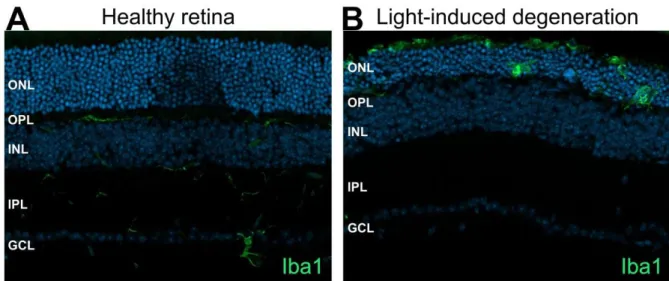
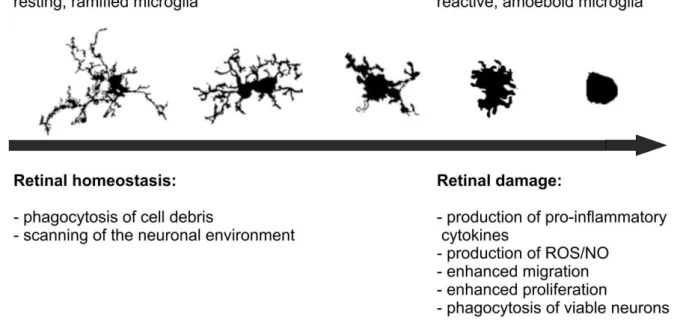
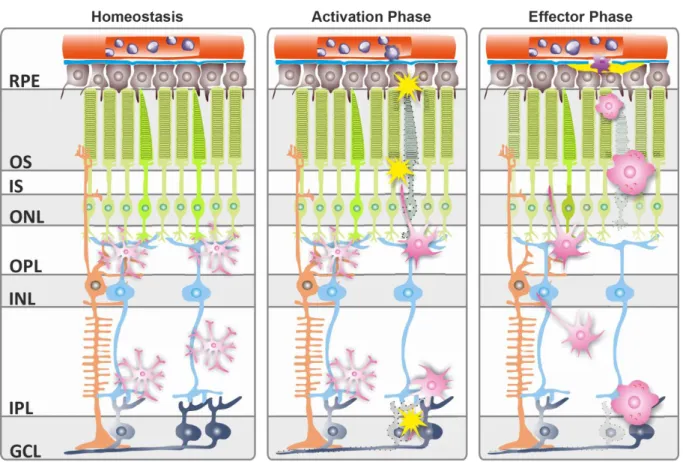
ÄHNLICHE DOKUMENTE
Previous studies have shown that microglia can promote tumor growth and invasion through various molecular and cellular mechanisms, one of which includes MT1-MMP
On the other hand, during tumor growth or therapies, different endogenous TLR ligands could be released by dying cancer cells or necrotic tissue to activate TLRs, this moderate
elegans counterparts in terms of sequence (let-7) or highly similar in terms of domain architecture (lin-28 and lin- 41), they are even interconnected in a conserved pathway
Even though the cell ultrastructure was preserved differently depending on the fixation method used, we were able to confirm our previous
Materials and Methods: Stem cell marker expression of CD133, CD44, C-KIT, CD34, ITGA6, OCT4, DDX5 and MELK in canine prostate carcinomas and prostate cyst cell lines were
Microglial cells expressing GFP (green) in the deep retinal layer, vessels are stained with lectin (red). bar =
Based on the quantitative analysis of the spatial growth distribution, and the specific transcript accumulation of FpXET1 in young elongating tissue, it is proposed that FpXET1 is
Besides the contribution of mast cells to allergic inflammation [132], they also seem to be involved in non-allergic inflammatory diseases of the gut, since in the intestine of