Gallium nitride has been successfully used for the production of blue and white light-emitting diodes (LEDs) for the last 30 years.
Volltext
Abbildung
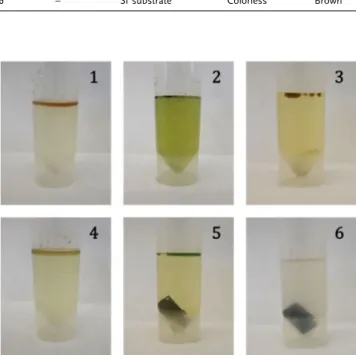
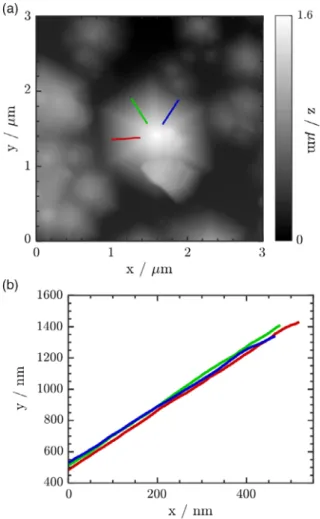
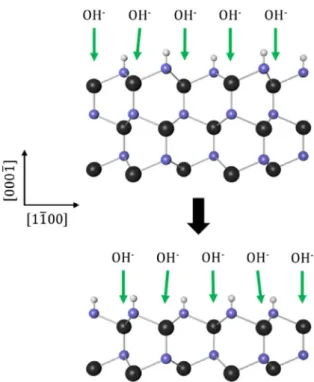
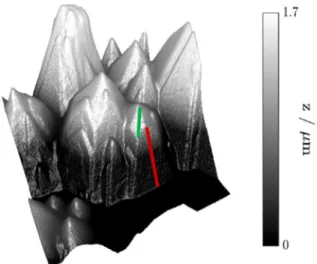
ÄHNLICHE DOKUMENTE
Reflection high-energy electron diffraction (RHEED) is established as a powerful tool in MBE, and is routinely used to investigate growth mode, growth rate, flatness, crystal
Speziell die drei letzten bilden eine thermodynami- sche Stabilitätsfolge; man erhält nämlich aus Per- chlorcyclooctatetraen (y-C8Cl8) je nach dem Grad des
schon diese Stellen scheinen mir genügend zu erweisen, daß es sich. im Suttanipäta um einen jnitw/a-Gürtel handelt, und
Between these two apparatuses a homogenous magnetic field is applied in y-direction, giving rise to a precession of the spin during the time of flight T from the first to the
It was quite dark when the door which communicated with the corridor opened, and Agmahd entered, followed by a young priest, who brought me food and a cup of some strange sweet-.!.
M.. Proposed models, algorithms.. c ) Mapping of economic damage. d ) Calculation of fields of pollutant maximum concentrations under normal un- favorable
The structure of the title complex appears to rep- resent an intermediate state between a fully ionic ex- treme with linearly two-coordinate silver in a cation [RNCAgCNR] +
Given the dramatic increase in artificial light in recent years, we see an urgent need for research on the physiological, human health, ecological, and