The changing roles of Hox3 genes in insect evolution: characterizing the zen paralogues in the beetle Tribolium castaneum
Volltext
Abbildung
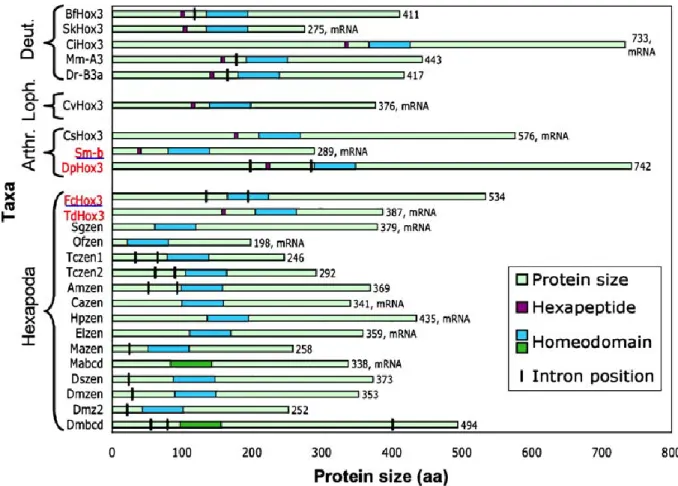
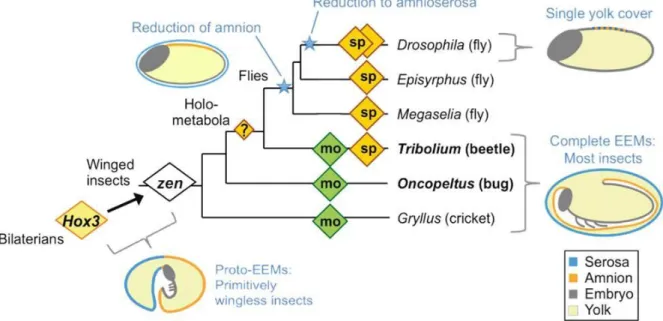
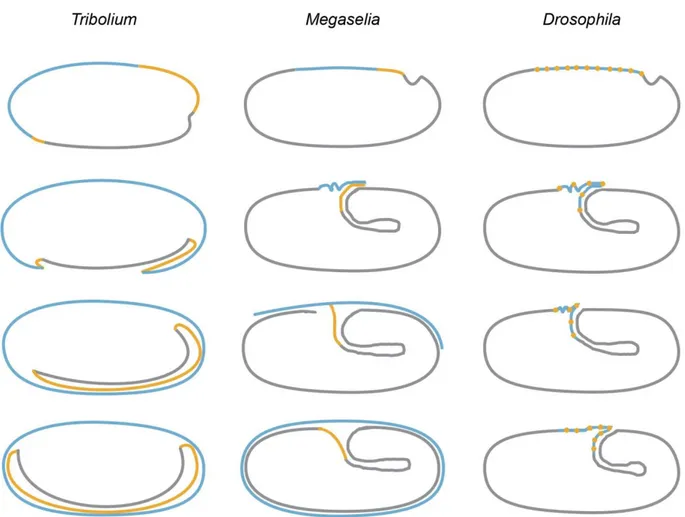
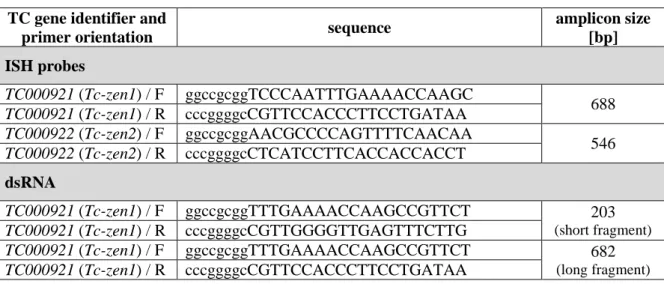
ÄHNLICHE DOKUMENTE
Figure 2.3 Possible signal transduction mechanisms of insect OSNs 10 Figure 2.4 The antennal lobe, the primary olfactory center in the insect brain 12 Figure 2.5 Current
Statistical test details of differences in the average cooperation level in the prisoner’s dilemma games of the static-network treatment (STATIC) and the three
In Drosophila Egfr signalling is required only late in development and its function is restricted to the tarsal region, but it is possible that the EGFR signalling pathway
GABA gamma-Aminobutyric acid GFP green fluorescent protein GL antennal lobe glomeruli GNG gnathal ganglia GOC gnathal olfactory center GR gustatory receptor GSN
In addition, I analyzed the following defined chromosomal rearrangements (e.g. Rong and Golic in Handler and James 2000): (i) inversions to test their properties as
The germ band now rotates in the egg for approximately 90° (Fig. Such a rotation has not been reported so far and it is unclear, whether just the imaged embryo behaved this way.
In order to identify the alkene biosynthetic mechanisms, the fatty acid profile was explored in glands and different developmental stages of Tribolium castaneum with
4) Generation of a specific anti-Sox15 antibody and expression analysis of Sox15 in different tissues and cell types was performed. 5) To understand the role of Sox15, we decided