Sustainable Hydrogenation of Nitroarenes, Alkenes and Alkynes.
Volltext
Abbildung
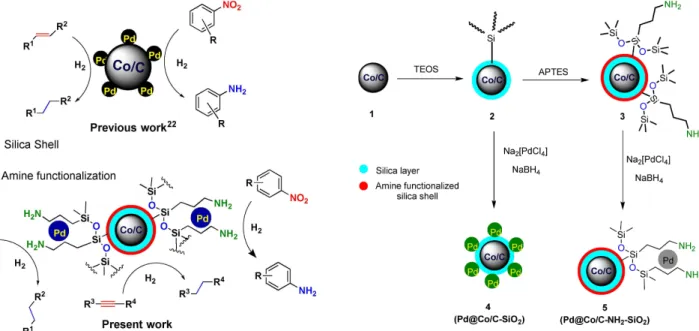
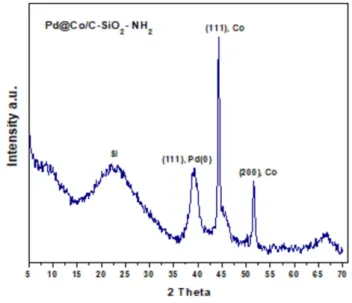
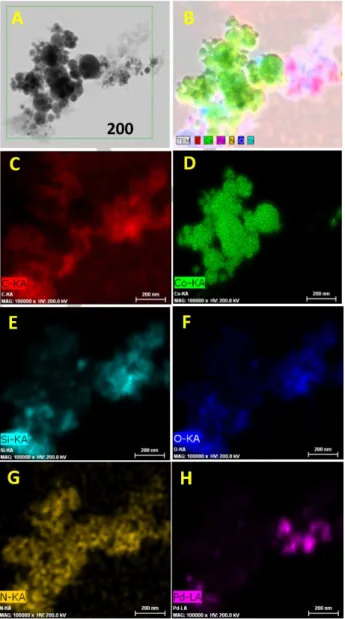
![Table 2. Substrate scope using Pd@Co/C SiO 2 NH 2 catalyst 5 [a] .](https://thumb-eu.123doks.com/thumbv2/1library_info/3732667.1508746/5.892.78.818.119.859/table-substrate-scope-using-pd-co-sio-catalyst.webp)
ÄHNLICHE DOKUMENTE
anisotropy of these exchange-coupled core-shell MNPs is mostly determined by the relative fraction of Co ferrite, whose increase determines a gradual transition to systems with
was prepared by dissolving 50 mg of MPA in 15 ml of methanol. The pH value of the stock solution was adjusted to 10.6 by addition of trimethylammonium hydroxide. To 9.5 mg of
Spin and orbital magnetic moments of deposited small iron clusters studied by x-ray magnetic circular dichroism spectroscopy. Size-dependant magnetism of deposited small iron
In this study the preferential intracellular localization of gold nanoparticles has been quantitatively evaluated by the stereological method of the relative deposition index
Water accessible porosity and water absorption coefficient of ultra-lightweight (left) and lightweight concretes (right) modified with 10 wt.% of silica fume (SF) and 1, 3, 5, and
As the measurements of the magnetic moment used for the calculation of the effective diffusion parameters start about half a day after the first exposure to air, the first reac- tion
[81] Dorota Napierska, Virginie Rabolli, Leen C J Thomassen, David Dinsdale, Catherine Princen, Laetitia Gonzalez, Katrien L C Poels, Micheline Kirsch-Volders, Dominique Lison, Johan
In both systems however, superconductivity is destroyed below a critical particle size, which is consistent with the Anderson criterion 2 , according to which superconductivity