Functional analysis of the role of GCN2 kinase in longevity and amino acid homeostasis in
Volltext
Abbildung


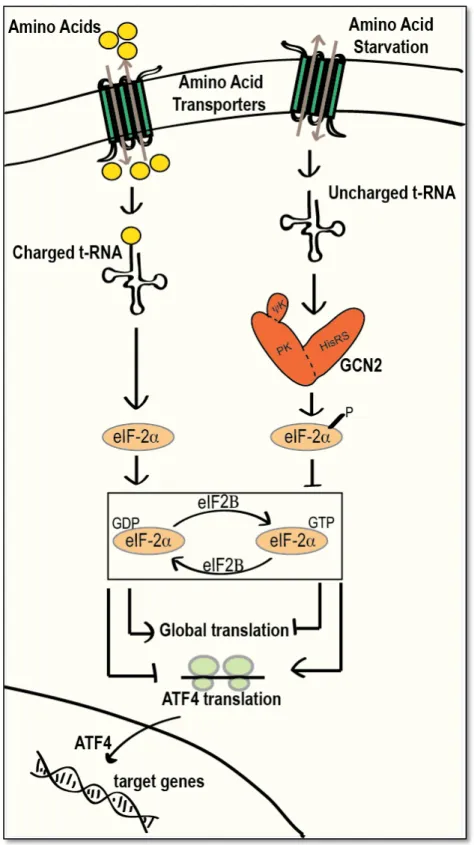
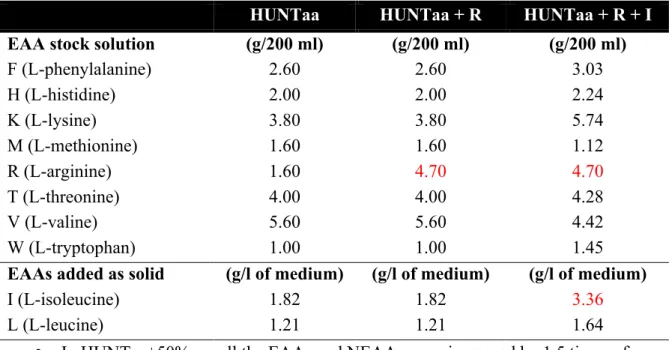
ÄHNLICHE DOKUMENTE
In humans, titin is encoded by a single gene on chromosome 2, region 2q31 (Labeit et al. In the skeletal and cardiac muscles, each titin molecule spans half a sarcomere from
The phosphorylation on Ser473 within a C-terminal hydrophobic motif leads to full activation of PKB and mediated by two members of the PI3K-related kinase (PIKK) family,
Based on the in vitro phosphorylation sites in the ribosomal protein Rpl24a one can deduce the minimal consensus sequence (K/R)XXS(K/R) for a potential substrate. To
Cell separation, the degradation of the septum after cytokinesis, requires the transcription of genes controlled by the Ace2 transcription factor (28. Cbk1
A, Protein levels of phosphorylated AKT (Thr 308 ) were measured by Western blotting in whole-cell lysates from LUHMES cultures treated with Fe 2 ⫹ /METH for 6 –36 h in the presence
Neither for FAK nor Paxillin any degradation was observed (Fig.. 6.4: Mb31 and Mb36 displace FAK from focal adhesions at low micromolar concentrations. A) GFP-FAK MEFs were seeded
Three major kinases involved in the signal transduction cascade that restarts meiosis, are Maturation Promoting Factor (MPF), Mitogen-Activated Proteinkinase (MAPK)
ROS are thought to promote atherosclerosis through a variety of mechanisms, including enhanced oxidation of lipoproteins (Steinberg 1997), activation of proinflammatory genes (Marui,