Polynuclear Group 13 Compounds: Synthesis, Characterization, and Reactivity
Volltext
Abbildung
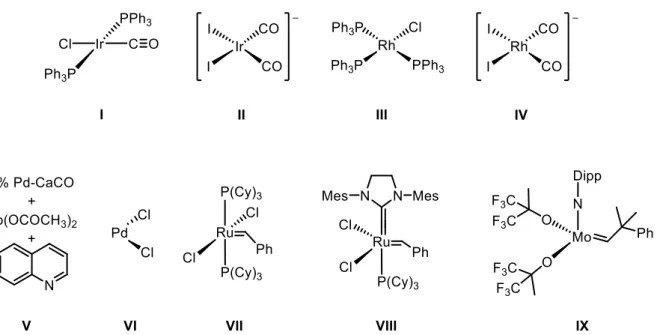
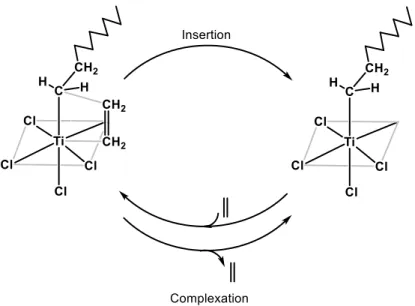
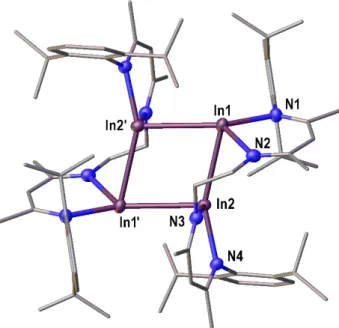
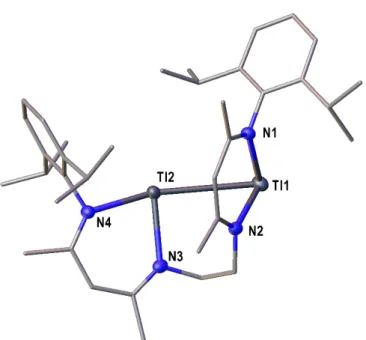
ÄHNLICHE DOKUMENTE
Although several syntheses of borazine derivatives using borazine as starting material are reported, bor- azine is not a suitable starting material for further syntheses due to
There are four inequivalent pro- tons and six inequivalent carbon atoms (some of them broadened) of the η 6 -bound cymene ligand giving res- onances in the 1 H resp. 13 C NMR
Institut f¨ur Anorganische Chemie, Julius-Maximilians-Universit¨at W¨urzburg, Am Hubland, 97074 W¨urzburg, Germany. Reprint requests
The aforementioned spectro- scopic data as well as the required twofold excess of BeCl 2 already indicate that [Pd(PCy 3 ) 2 ] does not form a Lewis base adduct with BeCl 2 in
A detailed analysis of the J 1 coupling taking into account the molecular structures of the three available heptanuclear com- plexes [Mn III 6 M III ] 3 + + + (M = Cr, Fe, Co)
As the chosen coupling scheme did not provide a reasonable reproduction of the experimental data, our next approach was to take into account a coupling of Mn III ions belonging
The Kamlet-Taft solvatochromic comparison method was used to separate and quantify these effects: An increase in the percentage of organic co- solvent in the medium enhances
This thesis focuses on the development of molecular mechanical (MM) methods and force fields to model hyper-valent molecules, transition metal complexes and ultimately, the study