Elucidation of the Intersystem Crossing Mechanism in a Helical BODIPY for Low-Dose Photodynamic Therapy
Volltext
Abbildung
![Table 1: Photophysical parameters of the compounds. [a]](https://thumb-eu.123doks.com/thumbv2/1library_info/3734453.1508886/3.892.80.435.83.400/table-photophysical-parameters-of-the-compounds-a.webp)
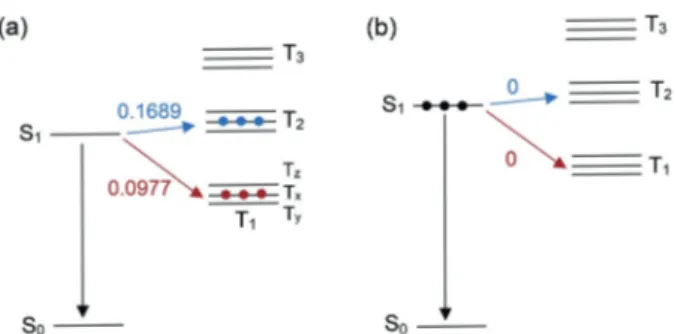
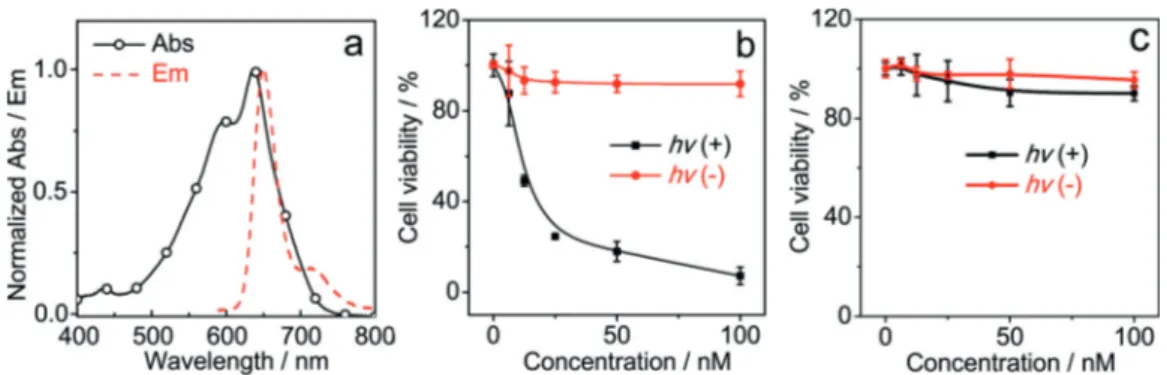
ÄHNLICHE DOKUMENTE
In conclusion, our results demonstrate that using a LD protocol on a 15-year-old CT scanner system in CTPA allows for a dose reduction of approximately 67% with similar subjective
Pharmacokinetics of GHB and detection window in serum and urine after single uptake of a low dose of GBL - an experiment with two volunteers.Drug Test
Objectives To analyze differences in variation of orthodontic diagnostic measurements on lateral cephalograms reconstructed from ultra low dose-low dose (ULD-LD) cone beam
The results of previous work showed the extraordinary potential of tetra-triethyleneoxysulfonyl substituted zinc phthalocyanine (ZnPc) as a novel PS for photodynamic cancer
The objectives were to determine quantitative liver function prospectively in patients with rheumatoid arthritis (RA) treated with low-dose methotrexate (MTX), to search for
No other risk factors for deterioration of lung volumes or gas exchange were found, including mean weekly MTX dose, age, gender, smoking, presence of rheumatoid factor and
Figure 4.8: Full treatment plan dose deposition using the model results to evaluate the lateral beam shape, displayed in CERR interface.. The geometry set up is a world of water of 10
The incubation of all tested glioblastoma cell lines with m-THPC/DMPC liposome formulation (in the absence of surfactant G1) slightly increased the value of