Separate functions of BTZ during post-transcriptional gene regulation
Volltext
Abbildung
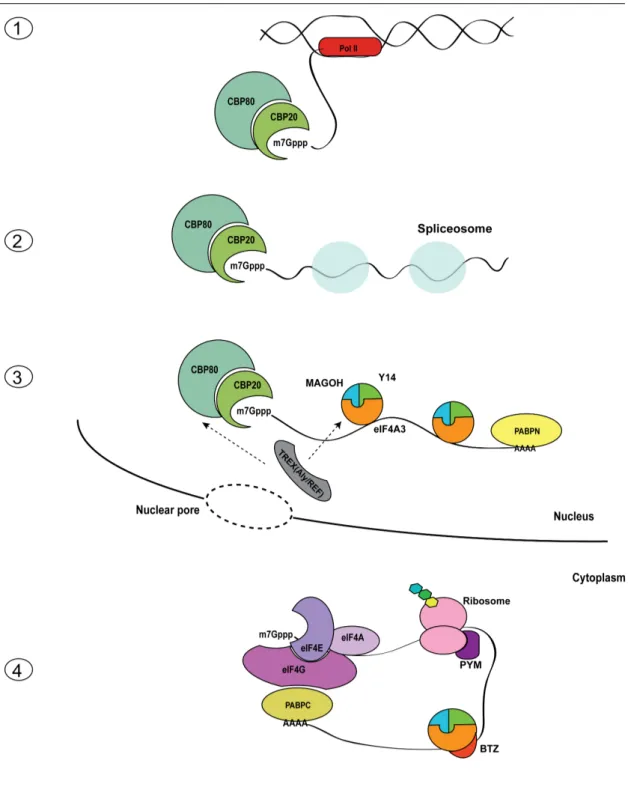
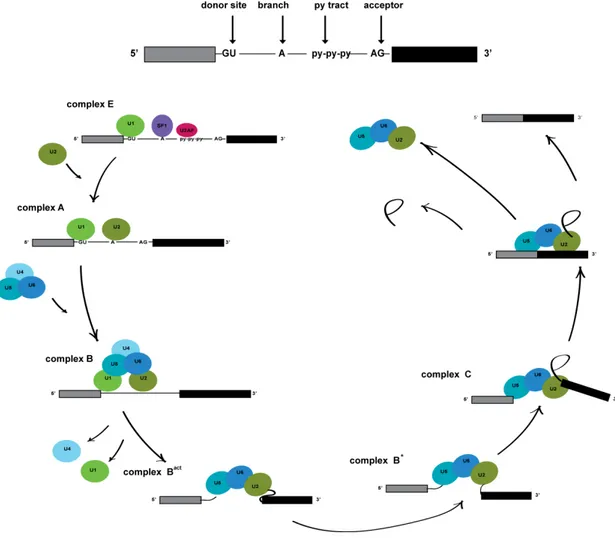
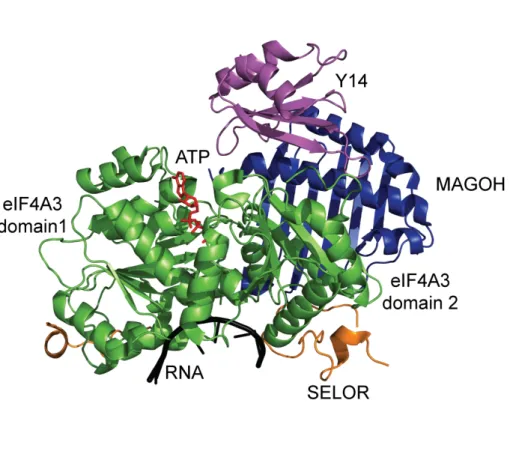
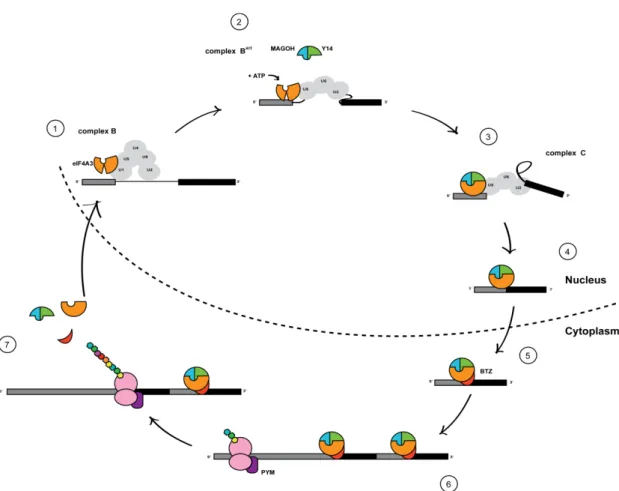
ÄHNLICHE DOKUMENTE
Die Bezugshöhe (BZH) ist die Straßenhöhe, die sich in der Mitte der Gebäudefas- sade des jeweiligen Vorhabens ergibt, wenn die im Bebauungsplan eingetrage- nen Straßenhöhen als
* Die Gruppenkennung sowie die Kassamarkt-ID werden von der Eurex Deutschland entsprechend der nachfolgenden Tabelle vergeben und dienen unter anderem der Festlegung
Es hat sich gezeigt, dass eine frühzeitige Einbindung der Personalvertretung für beide Seiten gewinnbringend ist und so ein Verständnis geschaffen wird, warum und wie der HPR
survival to adulthood and the prd mutant adult segmentation phenotype (Table 1; Fig. 3D), which is undistinguishable from wild-type (Fig. These results suggest that the prdRes
Der verunglückte Motorradfahrer blutete so stark, dass er im Krankenhaus weiter behandelt werden musste. Der Sohn erbte nach dem Tode seines Vaters so viel Geld, ohne dass er
Beeinträchtigungen von Natura 2000-Gebieten sowie die Gefahr schwerer Unfälle sind durch das Vorhaben nicht zu erwarten und es werden keine Vorhaben zuge- lassen, die einer Pflicht
c, The luciferase expression of HEK293 cells after transfection with the indicated luciferase reporter plasmid variants (Luc, Mot2 or M4) and mCherry-PAL under the control of
On top of the transcriptional regulation by the clock, circadian rhythms in mRNAs rely in large parts on post-transcriptional regulation, including alternative pre-mRNA splicing,